1..IntroductionInfrared (IR) lasers are ideally suited for the selective and precise removal of carious dental hard tissue while minimizing the loss of healthy tissue and patient discomfort.1 2 3 Since the initial investigations of Stern4 over 30 years ago, several unique laser dental applications have evolved for restorative dentistry, namely laser ablation of dental hard tissue,1 caries inhibition treatments by localized surface heating,5 and surface conditioning for bonding.8 The laser offers several potential advantages over the high-speed drill for the removal of dental hard tissue. The laser procedure is well tolerated and there is reduced or no pain due to diminished noise and vibration. Moreover, carious tissue can be preferentially removed due to the higher volatility of water and protein that are present in carious tissue at a higher ratio than in normal tissue. Lasers can be used to effectively modify the chemical composition of the remaining mineral phase of enamel. This is possible because the mineral, hydroxyapatite, found in bone and teeth contains carbonate inclusions that makes it highly susceptible to acid dissolution by organic acids generated from bacteria in dental plaque. Featherstone and Nelson5 demonstrated that transverse excited atmospheric pressure (TEA) CO 2 lasers operating at the 9.3–10.6 μm wavelengths could be used effectively to inhibit enamel demineralization. Upon heating to temperatures in excess of 400°C, the mineral decomposes to form a new mineral phase that has increased resistance to acid dissolution.5 Recent studies suggest that as a side effect of laser ablation, the walls around the periphery of a cavity preparation will be transformed through laser heating into a more acid resistant phase to have an enhanced resistance to future decay.6 7 Lasers can be tightly focused to drill holes for micropreparations with very high aspect ratio (depth/diameter), well beyond the capability of the dental drill which is limited by the size of the dental burr (Figure 1). This is of particular importance since early caries lesions are typically localized to the pit and fissures of the occlusal surfaces of the posterior dentition and these fissures are on the order of 200–300 μm wide. Therefore, lasers have the potential to substantially reduce the amount of tissue that needs to be removed for cavity preparations. An additional advantage of laser cavity preparation is that a smear layer of debris is not produced on the surface of the prepared cavity. Typically, in conventional cavity preparations using a drill, a smear layer is produced that has to be removed prior to sealing or bonding by acid etching. Thus, after laser removal, restorative materials can be applied directly to the ablated area without the necessity of further surface preparation.8 9 Figure 1The laser is well suited for the conservative removal of bacteria and carious enamel from the pits and fissures of the posterior dentition. (left and center) Laser pulses remove debris and decay (shaded areas) from fissure with 200–300 μm neck. If a water spray is not used during drilling then the walls have an enhanced resistance to decay and secondary caries (see Refs. 6 and 7). ![]() For several years medical lasers have been approved by the Food and Drug Administration for soft tissue vaporization. Only recently, however, have the first lasers been approved for hard tissue use in the US; those are the Er:YAG and the Er:YSGG lasers operating at 2.94 and 2.79 μm, respectively. The Er:YAG and Er:YSGG lasers efficiently ablate dental hard tissue, however they are expensive and somewhat limited in application, since they are not as well suited for caries prevention treatments as CO 2 lasers and are not applicable for soft tissue surgery due to poor hemostasis. A TEA CO 2 dental laser system operating at 9.6 μm is inherently much less expensive and more versatile. The CO 2 laser can operate at discrete wavelengths between λ=9 and 11 μm. Those wavelengths correspond to specific rotational–vibrational transitions in the ground state of gas phase CO 2 molecules. There are four principal vibrational emission bands which are centered at λ=9.3, 9.6, 10.3, and 10.6 μm, each consisting of several discrete rotational lines. The CO 2 laser has the highest gain at λ=10.6 μm, and all commercially available medical CO 2 lasers lase only at this wavelength. The CO 2 laser can be adapted to operate at the other wavelengths by various dispersive and nondispersive methods. Clinical dental ablation studies using a 9.6 μm radio-frequency (rf) excited CO 2 laser with a pulse duration of 70 μs have recently been initiated and are very promising.10 Excessive deposition of heat in the tooth may lead to eventual loss of pulpal vitality; thus any viable laser-dental procedure has to minimize the accumulation of heat in the tooth. The accumulation of heat in the tooth can be minimized by using a laser wavelength tuning to the maximum absorption coefficient of the tissue irradiated and by judicious selection of the laser pulse duration.11 In contrast to most other laser wavelengths, scattering is negligible in dental enamel at mid-IR wavelengths (λ=3–12 μm) and the energy deposition is determined by the absorption coefficient and the tissue reflectance. The two wavelength regions from 2.7 to 3.0 and 9 to 11 μm, offer the greatest potential for removal of hard tissue (Figure 2). Solid state erbium lasers (λ=2.94 and 2.79 μm) can be used to ablate enamel due to the strong water and OH − absorption of apatite. Er:YSGG laser emission is coincident with the narrow (OH − ) apatite absorption band at λ=2.8 μm, while Er:YAG emission overlaps the broad water absorption centered at λ=3 μm. Recently, absorption coefficients were measured for enamel at 9.3, 9.6, 10.3, and 10.6 μm wavelengths via combined measurements involving direct transmission through intact specimens of less than 50 μm thickness and time-resolved radiometry measurements in conjunction with simulations of heat conduction.12 These new values for CO 2 laser wavelengths are almost an order of magnitude lower than those previously reported based on angular resolved reflectance measurements and the Lorentz model.13 The absorption coefficient of dental enamel at 9.6 μm12 is 8000 cm −1 , which is approximately ten times higher than for the conventional λ=10.6 μm CO 2 laser wavelength used in medicine today and is markedly higher than for any other laser wavelengths throughout the visible and IR (see Figure 2). Near resonance to the phosphate absorption band of carbonated hydroxyapatite, the imaginary component of the refractive index increases markedly and the magnitude of the Fresnel reflectance exceeds 50 for normal incidence at the air/tissue interface. Therefore, the reflectance can be very high, 37 at 9.3 μm, 49 at 9.6 μm, and 13 at 10.6 μm.13 14 A similar rise in reflectance does not occur in dental enamel near resonance to the strong absorption band of water at 3 μm. Figure 2Infrared transmission spectrum through KBr pellet with 1 enamel powder. The molecular groups responsible for absorption are indicated with the relevant laser wavelengths. The wavelength, absorption coefficient (cm −1 ), absorption depth (1/e) , computed thermal relaxation time based on absorption depth, and tissue Fresnel reflectance () are indicated in the respective text boxes (see Refs. 12 13 14 46). ![]() The laser tissue interaction depends on the laser pulse duration, in addition to the absorption coefficient. For the suggested indications the optimum pulse duration should be on the order of the relaxation time for axial heat conduction τZ of the deposited energy in the tissue surface. This time constant is representative of the length of time required for heat diffusion from the layer of tissue heated by the laser, and depends on both the thermal diffusivity and the absorption coefficient at the respective laser wavelength. If the diameter of the laser beam is much greater than the depth of absorption, radial conduction can be neglected. The thermal diffusion or relaxation time for axial heat conduction is given by τZ=1/(4κμa 2) , where κ is the thermal diffusivity and μa is the absorption coefficient of the tissue.11 This time is the length of time required for the temperature of the surface layer of thickness (1/μa) to drop to approximately half of the initial intensity. For laser pulse durations greater than τZ, the laser energy is conducted away from the enamel surface into the interior of the tooth during the laser pulse, resulting in inefficient surface heating and possible pulpal damage. If the pulse duration is too short, then the deposited power density may be too high, causing the generation of a plasma in the plume of ablated material. This plasma shields the surface of the tissue from the tail end of the laser pulse and markedly reduces the efficiency of ablation, thereby restricting the maximum achievable ablation rate. This is what occurs with the conventional TEA CO 2 laser with a 0.2–4 μs pulse duration due to the initial gain switched spike (Figure 3). Figure 3The temporal profiles of the two Argus Photonics Group TEA lasers employed in this study. The conventional TEA CO 2 laser pulse (dotted line) (∼2 μs) and the long pulsed TEA CO 2 laser pulse (∼8 μs) on modified system (solid line) are shown above. ![]() Early results with the CO 2 laser for the ablation of dental hard tissue were discouraging because those studies used continuous wave lasers operating at λ=10.6 μm (Ref. 1) and extensive peripheral thermal and mechanical damage was reported. However, recent studies using pulsed CO 2 lasers have demonstrated that dental hard tissues can be efficiently ablated with minimal heat deposition in the tooth. Koort and Frentzen15 demonstrated that a TEA CO 2 laser (λ=10.6 μm) with pulse durations of 100–200 ns could be used to ablate dentin without carbonization and necrosis of surrounding tissue and without generating cracks. Lukac et al.;16 found that by increasing the pulse duration of a TEA CO 2 laser (λ=10.6 μm) from 0.1 to 1 μs the ablation rate increased 200–300. Krapchev et al.;17 achieved ablation efficiencies of approximately 0.1 to 0.2 mm 3 /J for enamel and dentin, respectively, using a transverse excited (TE) low pressure CO 2 laser (λ=10.6 μm) with a pulse duration of 20 μs. Ablation efficiencies exceed those measured for the Er:YAG (λ=2.94 μm)18 laser on enamel. Since the repetition rate of CO 2 lasers is not as limited by the physics of the lasing medium as is the case for the erbium laser solid state lasers systems, it is feasible that dental CO 2 lasers can be produced with drilling rates similar to those of the high speed dental drill, 0.1–1 mm 3 /s .19 Ertl and Mu¨ller19 showed that a TE CO 2 laser operating at λ=10.6 μm with pulse durations of 130 μs could ablate enamel and dentin with greater efficiency than with 20 μs and 100 ns pulses. The absorption coefficient at 10.6 μm was reported to be 850 cm −1 , which would place the thermal relaxation time in dental enamel for this wavelength at 80 μs, which is consistent with the above results.12 Melcer20 showed that practical ablation rates are attainable for a 10.6 μm TEA CO 2 laser by extending the pulse duration to approximately 10 μs. He measured an increase in the maximum ablation rate from 1 to 2 μm/pulse to over 10 μm/pulse for enamel ablation. The thermal relaxation time for enamel at a wavelength of 10.6 μm is ∼70–80 μs, almost 2 orders of magnitude longer than it is for 9.6 μm. Therefore, the utilization of laser pulses on the order of 10 μs at 10.6 μm does not offer any obvious advantage over longer pulses in the 50–100 μs range. In contrast, at 9.6 μm where the thermal relaxation time in enamel is 1–2 μs, we believe it is more appropriate to use a 9.6 μm long pulsed TEA laser for hard tissue use. Forrer et al.;21 found that the ablation efficiency of porcine rib bone was higher and the ablation thresholds markedly lower for λ=9.3 and 9.6 μm than for the 10.6 μm wavelength. Ertl and Mu¨ller19 reported similar results with enamel. Thus, the ablation efficiency of enamel and dentin should be higher at λ=9.6 μm than at the standard (λ=10.6 μm) CO 2 wavelength, resulting in reduced heat deposition in tooth for similar irradiation conditions. In this paper, we demonstrate that dental enamel can be thermally modified for caries prevention treatments using 9.6 μm TEA laser pulses with a fraction of the energy required at other laser wavelengths and pulse durations. By stretching the TEA laser pulse to 8 μs in duration enamel and dentin can be efficiently ablated with high single pulse ablation rates. 2..Materials and Methods2.1.Tissue IrradiationTwo sealed TEA lasers designed and manufactured by Argus Photonics Group, Jupiter, FL were used to irradiate the tissue samples with fluences of 0.1–100 J/cm 2 and energies from 0.1 to 80 mJ/pulse. The pulse durations were 2 and 8 μs in length (Figure 3) and the repetition rate was fixed at 1 Hz to avoid heat accumulation effects. The laser pulse temporal profiles shown in Figure 3 were measured at room temperature with a HgCdTe detector (Boston Electronics, Boston, MA). The temporal profiles of Figure 3 were integrated to indicate that 72 of the energy is partitioned in the first 600 ns, which is the full width of the gain-switched spike for the unmodified TEA laser pulse. In contrast, only 14 of the energy is contained in the first 600 ns for the stretched TEA laser pulse. The laser energy was measured and calibrated using laser calorimeters and the laser spot size was measured by both scanning a razor blade across the beam and by directly imaging the beam with a pyroelectric laser beam profilometer (Pyrocam I, Spirocon). The spot profile was single mode and fluences were defined using a Gaussian beam with a 1/e2 beam diameter. The laser beam was focused to spot sizes between 200 and 1000 μm. Human coronal dentin and enamel thin sections of 200 μm thickness were prepared using a hard tissue microtome from caries free, unerupted molars, and premolars. The sections were mounted before an IR pyroelectric detector (perforation sensor) and the number of pulses required to drill through each section were recorded. After perforation, an Olympus microscope with a maximum magnification of 500 times, interfaced to a digital charge coupled device camera and image analysis software, was used to determine the crater volume. The diameter of the crater was measured at three positions: surface, halfway through, and on the backside of the respective sections by scanning the image plane of the calibrated microscope, and fitted to two conic sections to determine the volumes of the perforation craters for each set of irradiation conditions. 2.2.Surface Dissolution Experiments and Fourier Transform Infrared (FTIR) SpectroscopyThe effectiveness of 9.6 and 10.6 μm laser radiation as a potential means for caries prevention, was assessed by irradiating 5×5 mm2 blocks of polished bovine enamel. The surfaces of the enamel blocks were first serial polished to a 1 μm finish with 6, 3, and 1 μm diamond suspensions. The samples were irradiated using a 400 μm spot size that was scanned across the sample using a Newport MM-2000 motion control system interfaced to LABVIEW 4.0 software from National Instruments to uniformly treat the entire surface. The surface was scanned every 100 μm for sufficient overlap of the surface and five pulses were delivered to each spot at a repetition rate of 1 Hz. After irradiation, an infrared microscope (XAD Plus, Laser Precision Analytical) with a 150×150 μm aperture attached to a Fourier transform spectrometer (Model RFX-30, Laser Precision Analytical) was used to acquire FTIR reflectance spectra of the surface of the irradiated bovine blocks and the control samples. The relative intensity of the carbonate bands located at 1450 and 1410 cm −1 was calculated by integrating the normalized spectra from 1200 to 1500 cm −1 . The carbonate loss was defined as one minus the ratio of the area of the irradiated enamel over that of the nonirradiated samples times a hundred. After spectra were taken the samples were mounted on high-density-polyethylene disks and placed in a reaction vessel containing a solution of 0.1 M acetic acid buffered to a pH of 4.5 Aliquots of the solution are collected for 20 min at 2 min intervals after immersion with continual stirring. The aliquots were subsequently analyzed for calcium and phosphate to determine the dissolution rate. Calcium was analyzed using atomic absorption and phosphate concentration photometrically using an ammonium molybdate colored complex that absorbs light at 820 nm. The technique(s) for evaluating surface dissolution and carbonate content via reflectance IR spectroscopy have been described previously.22 3..Results and Discussion3.1.Surface ModificationThe irradiation fluence thresholds that produced microscopically observable surface changes on enamel were determined for CO 2 laser radiation at 9.6 and 10.6 μm for the conventional TEA laser with a 2 μs pulse duration. There were laser induced surface changes on enamel at incident fluences above 0.5–1 J/cm 2 for 9.6 μm and approximately 2–3 J/cm 2 for 10.6 μm. With the appropriate adjustments due to reflectance losses, 49 at 9.6 μm and 13.5 at 10.6 μm, the difference in absorbed energy between the two wavelengths is significantly greater. The absorbed fluences are calculated to be 0.25–0.5 J/cm 2 for 9.6 μm and 2–3 J/cm 2 for 10.6 μm, demonstrating a factor of 6–8 times the difference in the amount of absorbed energy required for the surface modification of enamel using 2 μs CO 2 laser pulses between the two wavelengths. With regards to the pulse duration, the required energy for 2 μs pulses is markedly lower than the 3–4 J/cm 2 required for surface modification with 9.6 μm laser pulses of 100 μs duration that were previously employed in surface modifications studies.23 24 25 Morphological changes in enamel during short (<1 μs) pulsed CO 2 laser irradiation was first observed by Nelson et al.;26 They observed that very distinct morphological differences were induced in the mineral phase for each of the CO 2 wavelengths. Similar wavelength dependent morphological differences were not noted between 10.6 and 9.6 μm after irradiation after 2 μs TEA laser pulses. This discrepancy may be due to the fact that the previously reported study26 reflected differences in absorbed fluence and not in wavelength, since all the samples were irradiated at the same incident fluence level for each wavelength. The ablation thresholds vary markedly with wavelength due to the 40 difference in reflectance and the order of magnitude variation in the absorption coefficient between 9.6 and 10.6 μm. Moreover, 400 laser pulses were used in those previous studies and the periodic surface structures produced may have been unique to that particular laser mode structure due to extensive surface bombardment.26 During short pulsed laser irradiation without the addition of water as a coolant, dentin exhibited charring at 10.6 μm but not at the more highly absorbed 9.6 μm wavelength.27 The differences in the fluence threshold for ablation and the distinct differences noted in surface morphology between 9.6 and 10.6 μm are entirely consistent with the markedly different absorption depths and thermal relaxation times for those two wavelengths. It is interesting to note that with longer laser pulses, namely 100 μs, this difference does not manifest itself28 because the thermal diffusion length at 9.6 μm for a 100 μs laser pulse is about 10 μm, which is roughly equivalent to the absorption depth of 10–12 μm at λ=10.6 μm. 3.2.Infrared Spectroscopy and Caries Prevention StudiesFTIR was used to determine chemical changes in dental enamel as a result of laser irradiation. In previous studies, we discovered that FTIR spectroscopy in spectral reflectance mode was well suited for resolving chemical changes on the surface of enamel.22 29 30 The principal advantage of this technique is that the tissue reflectance is only influenced by a surface layer of a thickness on the order of the wavelength of the light. Thus, surface changes localized to the outer 10 μm of tissue are probed. The carbonate bands centered at 6–7 μm disappeared completely as a result of irradiation at fluences of approximately 0.5–1 J/cm 2 (Figure 4) with the (2 μs) TEA 9.6 μm CO 2 laser pulses. This is a four-to-eight fold reduction in the required energy as compared to the longer 100 μs pulses used in previous studies which required 4 J/cm 2 . While for the 10.6 μm laser pulses the reduction in the required fluence was less dramatic, the carbonate band disappeared at fluence levels of 3 J/cm 2 as compared to approximately 5–6 J/cm 2 for the longer 100 μs laser pulses a factor of 1.5–2 difference in fluence.29 Integration of the area of carbonate bands in the spectra enabled a relative carbonate loss to be calculated based on normalization to the carbonate band of the nonirradiated samples. In previous studies, we directly correlated the changes in the carbonate bands with the transformation of the carbonated hydroxyapatite mineral to the more acid resistant purer phase hydroxyapatite.22 The incident fluence that was sufficient to induce loss of carbonate in the FTIR spectra coincided with the optimum laser fluence that inhibited both surface and subsurface acid dissolution. Therefore, the FTIR is extremely useful as a rapid feedback nondestructive probe to elucidate the optimum laser parameters to inhibit acid dissolution. Figure 4The carbonate loss (solid symbols—left axis) determined using IR spectroscopy and the surface dissolution rate reduction (hollow symbols—right axis) relative to nonirradiated control samples for bovine enamel samples is plotted vs incident fluence after 9.6 μm (triangular symbols) and 10.6 μm (square symbols) (2 μs) TEA CO 2 laser irradiation. ![]() The results of the surface dissolution studies are shown graphically in Figure 4. The surface dissolution rate of the laser irradiated 5×5 mm 2 bovine blocks relative to nonirradiated control samples was determined for fluence levels up to 4.0 J/cm 2 for 10.6 and 9.6 μm laser irradiation. After irradiation with five 9.6 μm laser pulses of 2 μs duration in each spot at an incident fluence of 0.6 J/cm 2 /pulse (absorbed fluence of 0.3 J/cm 2 ), the rate of acid dissolution of enamel decreased by 40–50. The reduction in the relative surface dissolution rate closely paralleled the reduction in the carbonate band intensities, as was previously observed with longer 100 μs laser pulses.22 Significantly higher irradiation intensities were required to reduce the rate of acid dissolution by a similar amount after 10.6 μm laser irradiation, namely an incident fluence of 3.0 J/cm 2 and an absorbed fluence of 2.6 J/cm 2 . Moreover, the required irradiation intensities using the 2 μs TEA laser pulses are from five to ten times lower than those required using 9.6 μm laser pulses of a 100 μs duration, namely an incident fluence of 4–5 J/cm 2 .22 As indicated in the earlier section of this paper dealing with the problem of heat accumulation and thermal damage, the greatest potential danger of laser irradiation is the risk of excessive heat accumulation. Dental pulp, the soft tissue of the center of the tooth, is rigidly encased in a confined space enclosed by hard tissue, therefore it is extremely susceptible to permanent damage due to thermally induced inflammation.31 The 1965 Zach and Cohen study of the effect of heat on the pulp of Rhesus monkeys indicated that a temperature rise of 5.5°C in the pulp caused irreversible puplitis in 15 of the pulps.32 The accumulation of heat after use of the dental drill for cavity preparations can raise the pulpal temperatures to dangerous levels if air and/or water cooling are not used.33 Similarly, the use of multiple pulse irradiation without air/water cooling may also result in the accumulation of heat to levels dangerous to the pulp. Subsurface thermocouple measurements, simulations of heat conduction, and histological examinations during laser irradiation show that the extent of pulpal heating is determined by the rate of deposition of the laser energy in the tooth, the distance from the laser spot to the pulp, and the rate of energy loss from the tooth.34 35 36 37 By using the shorter laser pulse we are modifying a 1 mm spot size on the enamel surface with a total absorbed energy delivered of only 20–100 mJ for 5–25 laser pulses (0.5 J/cm 2 ) versus 100–2500 mJ in our previous studies utilizing 100 μs 9.6 μm laser pulses (2.5 J/cm 2 ). Note that other groups report the use of continuous wave CO 2 and argon ion lasers for caries prevention and they utilize absorbed fluences in the 100–200 J/cm 2 range, factors of 200–400 times higher than what we report in this study. The high fluences required for effective use of those systems may lead to excessive heat accumulation in the tooth.38 39 40 This study indicates that by using shorter laser pulses the energy needed to modify the surface of enamel for caries prevention can be reduced by a factor of 5–10 using short (<10 μs) 9.6 μm laser pulses. 3.3.Caries AblationAblation studies were performed at 9.6 and 10.6 μm with unmodified TEA laser pulses of 2 μs duration with the gain switched spike present in the first 100–200 ns of the pulse. The onset of a plasma initiated by the initial gain switched spike, prevented ablation at rates sufficient for clinical application. The rates were restricted to 2–3 μm/pulse for enamel and 6–8 μm/pulse for dentin for both 9.6 and 10.6 μm laser pulses.27 The pulse duration was subsequently lengthened to 8 μs, resulting in almost an order of magnitude reduction in the magnitude of the gain switched spike. This change in the temporal profile of the laser pulse raised the threshold for plasma formation (see Figure 3) and markedly increased the maximum achievable ablation rate. Enamel and dentin ablation rates and efficiencies as a function of the incident laser fluence for 8 μs, 9.6 μm CO 2 laser pulses are shown in Figures 5 and 6. These values are for the perforation of 200 μm thick sections without the application of a water spray. The spot size was 280 μm and the repetition rate was 2 Hz. The ablation rate saturates at a maximum rate of approximately 25 μm/pulse at fluences above 10–15 J/cm 2 . The peak enamel ablation efficiency at 9.6 μm with the 8 μs pulse approaches 0.1 mm 3 /J and is comparable to the highest values reported in the literature for either the longer pulsed CO 2 laser (20–300 μs) or the erbium laser systems. Maximum dentin ablation rates approached 50 μm/pulse at fluences of only 10–20 J/cm 2 and ablation efficiencies exceeded 0.1 mm 3 /J . Figure 5The ablation efficiency in (mm 3 /J) (circles) and the single pulse ablation rate (μm/pulse) (triangles) for perforation of 200 μm thick sections of human enamel. Each data point is the mean of three measurements ±standard deviation (s.d.). A dotted line is empirically drawn through the ablation rate data as a visual aid. It represents an increase in the ablation rate with increasing influence followed by saturation of the ablation rate above the plasma-shielding threshold. ![]() Figure 6The ablation efficiency (in mm 3 /J ) (circles) and the single pulse ablation rate (in μm/pulse) (triangles) for perforation of 200 μm thick sections of human dentin. Each data point is the mean of three measurements ± s.d. A dotted line is empirically drawn through the ablation rate data as a visual aid. It represents an increase in the ablation rate with increasing fluence followed by saturation of the ablation rate above the plasma-shielding threshold. ![]() The CO 2 laser couples more strongly to the mineral component of dental hard tissue than the Er:YAG laser and therefore it may not be as dependent on the requirement of the addition of a water spray to alleviate stalling problems such as those encountered with the erbium laser systems. We have been able to perforate 2 mm of enamel and dentin with relatively low fluences. The enamel ablation rates of 10–25 μm/pulse are lower than the longer pulsed CO 2 and Er:YAG laser systems which can ablate at rates exceeding 100 μm/pulse. However, high repetition rates are readily achievable for transverse excited CO 2 lasers and very high enamel and dentin removal rates are feasible. Studies have shown that pulsed lasers can generate strong compressive and tensile stress waves due to rapid laser heating.41 These shock waves propagate through the tissue radiating outward from the site of absorption. Generally, biological tissue has a relatively high compressive strength, however the tensile strength is much weaker and tensile forces can cavitate soft tissue and generate cracks in hard tissue. We have previously reported extensive mechanical damage peripheral to the respective ablation sites with Q-switched Er:YAG ablation42 and such cracks have been reported for high single pulse energy Er:YAG and Er:YSGG laser pulses.43 Similar cracks due to thermally induced stresses have also been produced in teeth using continuous wave CO 2 lasers.44 We did not observe any obvious stress related peripheral damage for either the conventional (2 μs pulse) or the long pulse (8 μs pulse) TEA laser systems. Ablation craters produced via perforation of human enamel and dentin are shown in Figure 7. The craters are extremely clean without any laser associated peripheral damage. Figure 7Holes drilled through 200 μm thick sections of enamel and dentin with λ=9.6 μm laser pulses of 8 μs pulse duration show no evidence of cracking and extended thermal damage. The image on the left was taken at 50 times magnification and it demonstrates the large number of holes that can be drilled through a thin tooth section without inducing any fractures. The image on the right shows the surface morphology of one of the holes produced in enamel at a higher magnification. The enamel is melted and there is a ring of fused enamel of 10 μm thickness. ![]() 4..ConclusionsThese studies demonstrate that sealed, long pulsed TEA CO 2 lasers can ablate dental enamel and dentin efficiently and at a practical rate to be used clinically. Moreover, these short pulses may be ideally suited for the irradiation of specific high risk areas of the dentition that are highly susceptible to tooth decay, such as early occlusal caries lesions (Figure 1), in order to reverse or prevent the progression of tooth decay in those areas. It was necessary to greatly reduce the fraction of energy disposed in the initial 600 ns spike to avoid plasma formation that restricts the ablation rate to 2 μm/pulse. This study shows that enamel ablation rates exceeding 25 μm/pulse are achievable with 9.6 μm sealed TEA lasers with a longer pulse duration of approximately 8 μs. TEA laser technology utilizes conventional direct current low frequency power supplies, which greatly reduces the intrinsic cost of these devices. In addition, the laser can be air cooled because of low energy requirements. Many of the current pulsed CO 2 lasers operating for industrial or medical application utilize rf power sources which are more expensive to produce. Moreover, rf CO 2 laser systems are not well suited for the efficient production of laser pulses with pulse durations as short as a few microseconds. Er:YAG and Er:YSGG lasers are inherently expensive due to the cost of the laser medium itself and the high single pulse energies required for drilling, namely greater than a 100 mJ/pulse. Another advantage of CO 2 laser technology in general over current erbium technology is that the peak repetition rate is not limited by the gain media to 10–30 Hz. Repetition rates in the kHz range have been achieved with TEA CO 2 lasers. Recently, Ivanenko and Hering45 demonstrated that high cutting rates in bone are possible without peripheral thermal damage using a 300 Hz mechanically Q-switched CO 2 laser. Thus, if higher cutting speeds are desired the laser beam can always be rapidly scanned at a high ablation rate.46 AcknowledgmentsThis work was supported by research Grant Nos. NIH/NIDCR R41DE12971, R29DE12091, and R01E00958. The two TEA CO 2 lasers were provided by Argus Photonics Group. REFERENCES
H. A. Wigdor
,
J. T. Walsh
,
J. D. B. Featherstone
,
S. R. Visuri
,
D. Fried
, and
J. L. Waldvogel
,
“Lasers in Dentistry,”
Lasers Surg. Med. , 16 103
–133
(1995). Google Scholar
W. Seka
,
J. D. B. Featherstone
,
D. Fried
,
S. R. Visuri
, and
J. T. Walsh
,
“Laser ablation of dental hard tissue: From explosive ablation to plasma-mediated ablation ,”
Proc. SPIE , 2672 144
–158
(1996). Google Scholar
R. H. Stern
and
R. F. Sognnaes
,
“Laser beam effect on hard dental tissues,”
J. Dent. Res. , 43 873
(1964). Google Scholar
J. D. B. Featherstone
and
D. G. A. Nelson
,
“Laser effects on dental hard tissue,”
Adv. Dent. Res. , 1
(1), 21
–26
(1987). Google Scholar
N. Konishi
,
D. Fried
,
J. D. B. Featherstone
, and
M. Staninec
,
“Inhibition of secondary caries by
CO2
laser treatment,”
Am. J. Dent. , 12
(5), 213
–216
(1999). Google Scholar
D. A. Young
,
D. Fried
, and
J. D. B. Featherstone
,
“Ablation and caries inhibition of pits and fissures by IR laser irradiation,”
Proc. SPIE , 3910 247
–253
(2000). Google Scholar
L. F. Cooper
,
M. L. Myers
,
D. G. A. Nelson
, and
A. S. Mowery
,
“Shear strength of composite resin bonded to laser pretreated dentin,”
Prosth. Dent., 60 45
–49
(1988). Google Scholar
S. R. Visuri
,
J. L. Gilbert
, and
J. T. Walsh
,
“Shear test of composite bonded to dentin: Er:YAG laser vs. dental hand piece preparations,”
Proc. SPIE , 2394 223
–227
(1995). Google Scholar
H. Wigdor
,
J. T. Walsh
, and
R. Mostofi
,
“The effect of the
CO2
laser (9.6 μm) on the dental pulp in humans,”
Proc. SPIE , 3910 158
–163
(2000). Google Scholar
M. J. C. van Gemert
and
A. J. Welch
,
“Time constants in thermal laser medicine,”
Lasers Surg. Med. , 9 405
–421
(1989). Google Scholar
M. J. Zuerlein
,
D. Fried
,
J. D. B. Featherstone
, and
W. Seka
,
“Optical properties of dental enamel at 9–11 μm derived from time-resolved radiometry,”
IEEE J. Sel. Top. Quantum Electron. , 5
(4), 1083
–1089
(1999). Google Scholar
G. Duplain
,
R. Boulay
, and
P. A. Belanger
,
“Complex index of refraction of dental enamel at
CO2
wavelengths,”
Appl. Opt. , 26 4447
–4451
(1987). Google Scholar
D. Fried
,
R. E. Glena
,
J. D. B. Featherstone
, and
W. Seka
,
“Permanent and transient changes in the reflectance of
CO2
laser irradiated dental hard tissues at λ=9.3, 9.6, 10.3, and 10.6 μm and at fluences of 1–20
J/cm2
,”
Lasers Surg. Med. , 20 22
–31
(1997). Google Scholar
M. Lukac
,
F. Hocevar
,
S. Cencic
,
K. Nemes
,
U. Keller
,
R. Hibst
,
D. Sustercic
,
B. Gaspirc
,
U. Skaleric
, and
N. Funduk
,
“Effects of pulsed
CO2
and Er:YAG lasers on enamel and dentin,”
Proc. SPIE , 1880 169
–175
(1993). Google Scholar
V. B. Krapchev
,
C. D. Rabii
, and
J. A. Harrington
,
“Novel
CO2
laser system for hard tissue ablation ,”
Proc. SPIE , 2128 341
–348
(1994). Google Scholar
G. B. Altshuler
,
A. V. Belikov
, and
A. V. Erofeev
,
“Laser treatment of enamel and dentin by different Er-lasers ,”
Proc. SPIE , 2128 273
–281
(1994). Google Scholar
T. Ertl
and
G. Muller
,
“Hard tissue ablation with pulsed
CO2
lasers,”
Proc. SPIE , 1880 176
–181
(1993). Google Scholar
M. Forrer
,
M. Frenz
,
V. Romano
,
H. J. Altermatt
,
H. P. Weber
,
A. Silenok
,
M. Istomyn
, and
V. I. Konov
,
“Bone-ablation mechanism using
CO2
lasers of different pulse duration and wavelength,”
Appl. Phys. B: Photophys. Laser Chem. , 56 104
–112
(1993). Google Scholar
J. D. B. Featherstone
,
D. Fried
, and
C. Duhn
,
“Surface dissolution kinetics of dental hard tissue irradiated over a fluence range of 1–8
J/cm2
at a wavelength of 9.3 μm,”
Proc. SPIE , 3248 146
–151
(1998). Google Scholar
J. D. B. Featherstone
,
N. A. Barrett-Vespone
,
D. Fried
,
Z. Kantorowitz
, and
J. Lofthouse
,
“
CO2
laser inhibition of artificial caries-like lesion progression in dental enamel,”
J. Dent. Res. , 77
(6), 1397
–1403
(1998). Google Scholar
J. D. B. Featherstone
,
N. A. Barrett-Vespone
,
D. Fried
,
Z. Kantorowitz
,
J. Lofthouse
, and
W. Seka
,
“Rational choice of
CO2
laser conditions for inhibition of caries progression,”
Proc. SPIE , 2394 57
–67
(1995). Google Scholar
J. D. B. Featherstone
,
D. Fried
,
S. M. McCormack
, and
W. Seka
,
“Effect of pulse duration and repetition rate on
CO2
laser inhibition of caries,”
Proc. SPIE , 2672 79
–87
(1996). Google Scholar
D. G. A. Nelson
,
J. S. Wefel
,
W. L. Jongebloed
, and
J. D. B. Featherstone
,
“Morphology, histology and crystallography of human dental enamel treated with pulsed low energy IR laser radiation,”
Caries Res. , 21 411
–426
(1987). Google Scholar
D. Fried
,
M. W. Murray
,
J. D. B. Featherstone
,
M. Akrivou
,
K. M. Dickenson
,
C. Duhn
, and
O. P. Ojeda
,
“Dental hard tissue modification and removal using sealed TEA lasers operating at λ=9.6 μm,”
196
–203
(1999). Google Scholar
S. M. McCormack
,
D Fried
,
J. D. B. Featherstone
, and
W. Seka
,
“Scanning electron microscope observations of
CO2
laser effects on dental enamel,”
J. Dent. Res. , 74
(10), 1702
–1708
(1996). Google Scholar
J. D. B. Featherstone
,
D. Fried
, and
E. R. Bitten
,
“Mechanism of laser induced solubility reduction of dental enamel,”
112
–116
(1997). Google Scholar
J. D. B. Featherstone
,
S. Pearson
, and
R. Z. LeGeros
,
“An IR method for quantification of carbonate in carbonated-apatites,”
Caries Res. , 18 63
–66
(1984). Google Scholar
H. E. Goodies
and
R. J. Rosenberg
,
“Histologic response of the pulp tissue to temperature probe and cavity preparation in the M. Fascicularis Monkey,”
Oral Surgery, 72 105
–107
(1991). Google Scholar
L. Zach
and
G. Cohen
,
“Pulp response to externally applied heat,”
Oral. Surg. Oral. Med. Oral. Pathol., 19 515
–530
(1965). Google Scholar
A. I. Hamilton
and
I. R. H. Kramer
,
“Cavity preparation with and without waterspray: Effects on the human dental pulp and additional effects of further dehydration of the dentine,”
Br. Dent. J. , 126 281
–285
(1967). Google Scholar
J. M. White
,
H. E. Goodis
, and
T. E. Daniels
,
“Effects of Nd:YAG laser on pulps of extracted teeth,”
Lasers Life Sci., 4
(3), 191
–200
(1991). Google Scholar
J. M. White
,
H. E. Goodis
,
K. T. Tran
,
W. Ho
, and
J. J. Kuddler
,
“In vivo pulpal response to Nd:YAG laser on dentin,”
J. Dent. Res. , 73 318
(1994). Google Scholar
D. Yu
,
J. L. Fox
,
J. Hsu
,
G. L. Powell
, and
W. I. Higuchi
,
“Computer simulation of surface temperature profiles during
CO2
laser irradiation of human enamel,”
Opt. Eng. , 32 298
–305
(1993). Google Scholar
A. Sagi
,
T. Segal
, and
J. Dagan
,
“A numerical model for temperature distribution and thermal damage calculations in teeth exposed to a
CO2
laser,”
Math. Biosci. , 71 1
–17
(1984). Google Scholar
G. L. Powell
,
W. I. Higuchi
,
J. L. Fox
,
D. Yu
, and
R. Blankenau
,
“Argon laser effect on demineralization of human enamel,”
Proc. SPIE , 1643 334
–379
(1992). Google Scholar
J. Hsu
,
J. L. Fox
,
Z. Wang
,
G. L. Powell
,
M. Otzuka
, and
W. I. Higuchi
,
“Combined effects of laser irradiation/solution fluoride on enamel demineralization,”
J. Clin. Laser. Med. Surg. , 16 93
–105
(1998). Google Scholar
J. L. Fox
,
D. Yu
,
M. Otsuka
,
W. I. Higuchi
,
J. Wong
, and
G. L. Powell
,
“Initial dissolution rate studies on dental enamel after
CO2
laser irradiation,”
J. Dent. Res. , 71 1389
–1397
(1992). Google Scholar
R. S. Dingus
and
R. J. Scammon
,
“Gru¨neisen-stress induced ablation of biological tissue ,”
Proc. SPIE , 1427 45
–54
(1991). Google Scholar
D. Fried
,
R. Shori
, and
C. Duhn
,
“Backspallation due to ablative recoil generated during Q-switched Er:YAG ablation of dental enamel,”
Proc. SPIE , 3248 78
–85
(1998). Google Scholar
G. B. Altshuler
,
A. V. Belikov
,
A. V. Erofeev
, and
R. C. Sam
,
“Optimum regimes of laser destruction of human tooth enamel and dentin ,”
Proc. SPIE , 1880 101
–107
(1993). Google Scholar
R. F. Boehm
,
J. Rich
,
J. Webster
, and
S. Janke
,
“Thermal stress effects and surface cracking associated with laser use on human teeth,”
J. Biomech. Eng. , 99 189
–194
(1977). Google Scholar
M. M. Ivanenko
and
P. Hering
,
“Wet bone ablation with mechanically Q-switched high-repetition-rate
CO2
,”
Appl. Phys. B: Lasers Opt. , 67 395
–397
(1998). Google Scholar
D. Fried
,
M. Zuerlein
,
J. D. B. Featherstone
,
W. D. Seka
, and
S. M. McCormack
,
“IR laser ablation of dental enamel: mechanistic dependence on the primary absorber,”
Appl. Surf. Sci. , 127 41
–50
(1997). Google Scholar
|
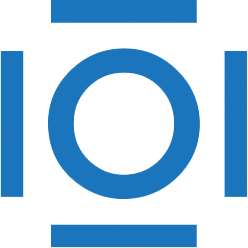
CITATIONS
Cited by 67 scholarly publications.
Gas lasers
Laser ablation
Pulsed laser operation
Carbon monoxide
Laser tissue interaction
Absorption
Dental caries